The acceptable level of inspired carbon dioxide (CO2) in diving gear is a controversial topic. Some current standards1,2 permit up to 20 mBar (2 kPa, 2% SEV) as the average inspired CO2 partial pressure, while some research, for example, work by the authors of this article,3,4 indicates that amount to be dangerous. Since submariners tolerate inspired CO2 levels that are higher than the current limits for diving gear, one could be forgiven for suspecting a marketing ploy by any manufacturer touting benefits of lower inspired CO2. A look at the physiology of CO2 shows, though, that the danger of high CO2 in diving is real and important. (Contamination with carbon monoxide
Effects of elevated CO2 partial pressure in the blood
CO2 usually influences breathing so that the body maintains a healthy arterial CO2 partial pressure (PaCO2) of approximately 40 Torr (40 mm Hg, 5.3 kPa) even when inspired gas contains a low concentration of CO2. However, the use of breathing gear is an unusual condition that is rarely covered in physiology courses.
What is partial pressure?
The concept of partial pressure of any gas, say “gas A”, in a cylinder of mixed gas is relatively straightforward: it is the pressure that would remain if all gas molecules that are not “gas A” were removed. (Figure 1a) (We assume an ideal gas, that is to say, we consider interactions among gas molecules to be insignificant.) Partial pressure of A indicates the number of molecules of A per volume. Numerically it is the product of total pressure and the fraction of the gas that consists of molecules A. (For example, the partial pressure of A in a gas containing 65% of A at a total pressure of 2 bar is 0.65 x 2 = 1.3 bar.) This also applies to containers at ambient pressure, for example, the lungs.
The meaning of partial pressure of a gas in a liquid is less obvious. The molecules in a gas, always in motion, exert pressure by bumping into the walls of their container. If one of those “walls” is a liquid surface, soluble gas molecules will dissolve in the liquid. The molecules in the liquid are also in motion, and molecules of gas reaching the surface again are able to leave the liquid. After “long enough” with a gas containing gas A in contact with the liquid, the rate at which molecules of A leave the liquid phase matches the rate at which they enter it. (Figure 1b) In other words, the gas and liquid are at equilibrium. The direction and rate of gas transfer of A across a liquid – gas surface surface depends on the partial pressure difference, gas to liquid. Although the number of molecules of gas A dissolved in a volume of the liquid depends also on the solubility of A in the liquid, it is useful to express the amount of dissolved gas A as the partial pressure of A in the gas at equilibrium with the liquid.
Figure 1. Illustrations of the concept of partial pressure a) in gases and b) in liquids.
a) Half of the molecules in the first gas cylinder are “gas A”. The partial pressure of A in the cylinder is thus half the total pressure, as indicated by the pressure gauge on the hypothetical second cylinder from which all molecules other than gas A have been removed.
b) The space above a liquid contains molecules of gas A, and gas A is soluble in the liquid. At equilibrium, one molecule of A leaves the liquid for each molecule of A that enters it, and the partial pressure of A in the liquid is defined as the partial pPressure of A in the gas in equilibrium with it.
Which partial pressures are important for whole-body CO2 balance?
The partial pressure of CO2 in arterial blood (PaCO2) is the value the body needs to control. In normal lungs, alveolar CO2 partial pressure (PACO2) (Figure 2) approximately equals PaCO2, as is discussed later in this article Finally, the gas exhaled at the end of a normal (“tidal”) breath, called “end tidal gas”, comes from the alveoli. Thus, the end tidal partial pressure of CO2 (PETCO2) approximates PACO2. Although PaCO2, PACO2 and PETCO2 are not identical because of regional and breathing cycle variations within the lungs, for the purposes of this article, they are equivalent.
Figure 2. The CO2 partial pressures discussed.
Blood in the lungs is exposed to alveolar gas with PACO2. The blood that leaves the lungs, usually equilibrated with alveolar gas, becomes the systemic arterial blood after its passage through the heart, with CO2 partial pressure PaCO2. PCO2 at the mouth and nose, shown as a function of time in the graph, varies from zero during inspiration to a maximum during expiration. The value at the end of a normal “tidal” breath, the “end tidal” value PETCO2, is a sample of mixed PACO2.
Effects of increased PaCO2
Elevated PaCO2 is called hypercapnia, or sometimes hypercarbia. Hypercapnia has these interrelated effects:
1. High PaCO2 causes changes in brain activity detectable by EEG, changes that impair both the ability to think clearly and the control of small muscles. EEG changes indicating early narcotic effects were measured when PETCO2 was elevated from approximately 40 to about 50 Torr.5 Changes in ability to think were detected with PETCO2 at or above 51 Torr.6 Hand steadiness decreased when PETCO2 was greater than about 47 Torr.7
In a study of CO2 effects with heavy exercise underwater,4 one diver whose PETCO2 reached about 52 Torr was unable to remember that the target pedal speed on the in-water cycle ergometer was 60 rpm, even though the tachometer showed the numbers in green for 58 to 62 rpm and in red for any other pedalling rate. Another diver with PETCO2 of approximately 65 Torr at the end of his dive seemed dazed after surfacing. Neither of those divers admitted to any symptoms of hypercapnia.
2. Very high PaCO2 has a narcotic or sedative effect. A published report gives details of two incidences:8 A diver exercising underwater with high breathing resistance became confused and irrational with PETCO2 of about 72 Torr. He refused to stop exercise, and later lost consciousness with PETCO2 near 90 Torr. Another diver in the same study maintained PETCO2 between 65 and 68 Torr for more than 20 minutes, then became confused.
3. High PaCO2 activates the “fight or flight” response, which, as well as affecting hormone levels, generates anxiety and irritability in some people. In the previously mentioned study with heavy exercise in the water, 4 an experienced Navy diver described a sudden sense of panic as his PETCO2 climbed from about 60 to 65 Torr. He found it difficult to control himself enough to surface in an orderly way. In an earlier study of effects of CO2 in the water,9 PETCO2 for one diver was seen to be approximately 51 Torr and climbing. The diver said that he felt fine, then surfaced suddenly, too fast and without proper communication.
4. High PaCO2 causes small blood vessels (arterioles), most
notably those in the brain and the skin, to expand. In the skin, the increase in blood flow causes flushing and a feeling of unpleasant heat. The diver mentioned above who experienced sudden panic 4 also reported that he felt very hot in the face.
Even though brain blood flow stays constant over a wide range of blood pressures, it is very sensitive to PaCO2; with PETCO2 of 50 Torr, cerebral blood flow increases by 40 to 50% over normal.5 (Figure 3) If blood outflow cannot keep up with inflow, pressure inside the skull increases. This may cause a throbbing, migraine-like headache. In the in-water study with heavy exercise,4 PETCO2 was greater than 50 Torr in the last minute of 23 person-dives, and divers reported headache after 12 of those dives.
Divers who breathe gas with elevated oxygen partial pressure and have increased brain blood flow also have increased oxygen delivery to the brain. This elevates the risk of central nervous system (CNS) oxygen toxicity at oxygen partial pressures normally considered safe.10
Figure 3. Effect of CO2 on brain blood flow.
a) blood flow as a function of blood pressure.
b) modulation of blood flow by PaCO2. The figures approximate textbook values. Magnitudes are correct, but the curves do not represent data.
5. High PaCO2 causes some people (but not everyone) to feel short of breath. Unfortunately, many people are totally unaware that their PaCO2 is elevated when they are diving; in the in-water study4 quoted above, approximately 40% of the divers with PETCO2 greater than 51 Torr denied having any symptoms. The lack of symptoms does not mean a lack of other effects.
An elevated PaCO2 isn’t healthy. One report indicates that that 17 of 181 civilian rebreather deaths between 1998 and 2010 were related to CO2 retention.11
Note that some effects of elevated PaCO2 take some time to wear off. The diver who seemed dazed4 remained so for 10 to 15 minutes. Headaches may last hours. Removing the source of the problem will help, but the improvement may not be immediate.
How or why can PaCO2 climb?
CO2 accumulates in the body if it is produced faster than it is cleared. Let’s examine this systematically.
CO2 Production
Metabolism is the primary source of CO2 in our bodies. When we convert carbohydrate into energy, ten molecules of CO2 are produced for every ten molecules of oxygen (O2) consumed. When we burn fat, the ratio is seven molecules of CO2 for every ten molecules of O2. Under normal resting conditions, we consume both energy sources, and the ratio is eight molecules of CO2 for every ten molecules of O2. The blood transports CO2 away from working tissue and dumps it into lung gas, where it also picks up O2 from lung gas to supply the working tissues.
The hypothetical 70-kg person at rest consumes about 250 mL O2 /min and produces about 200 mL CO2/min, where the gas volume is expressed at standard temperature and pressure (STP), that is, 0 °C and 1 bar (101.3 kPa, 760 Torr). Those rates can increase more than ten-fold when the person exercises.
Transport of O2 and CO2 in the blood
O2 is not very soluble in blood. Unless the oxygen partial pressure is very high (> 2 bar), O2 is only carried effectively attached to haemoglobin inside red cells. Each haemoglobin molecule can hold four molecules of oxygen. Oxygen transport capacity is limited by haemoglobin concentration and blood volume – when all the haemoglobin is “full” (100% saturated), no more O2 molecules can enter the blood.
CO2 is more soluble in blood than O2 is, but still only about 5% of the CO2 in blood is transported as dissolved gas. Another 5 to 10% travels attached to proteins in hemoglobin (not to the oxygen transport sites). Most of the CO2 in blood is carried inside the red cells as bicarbonate ion (HCO3–), because:
CO2 + H2O ↔ H+ + HCO3–.
The amount of CO2 that blood can hold is unlimited — except that extremely high PaCO2 would be fatal!
CO2 elimination in the lungs
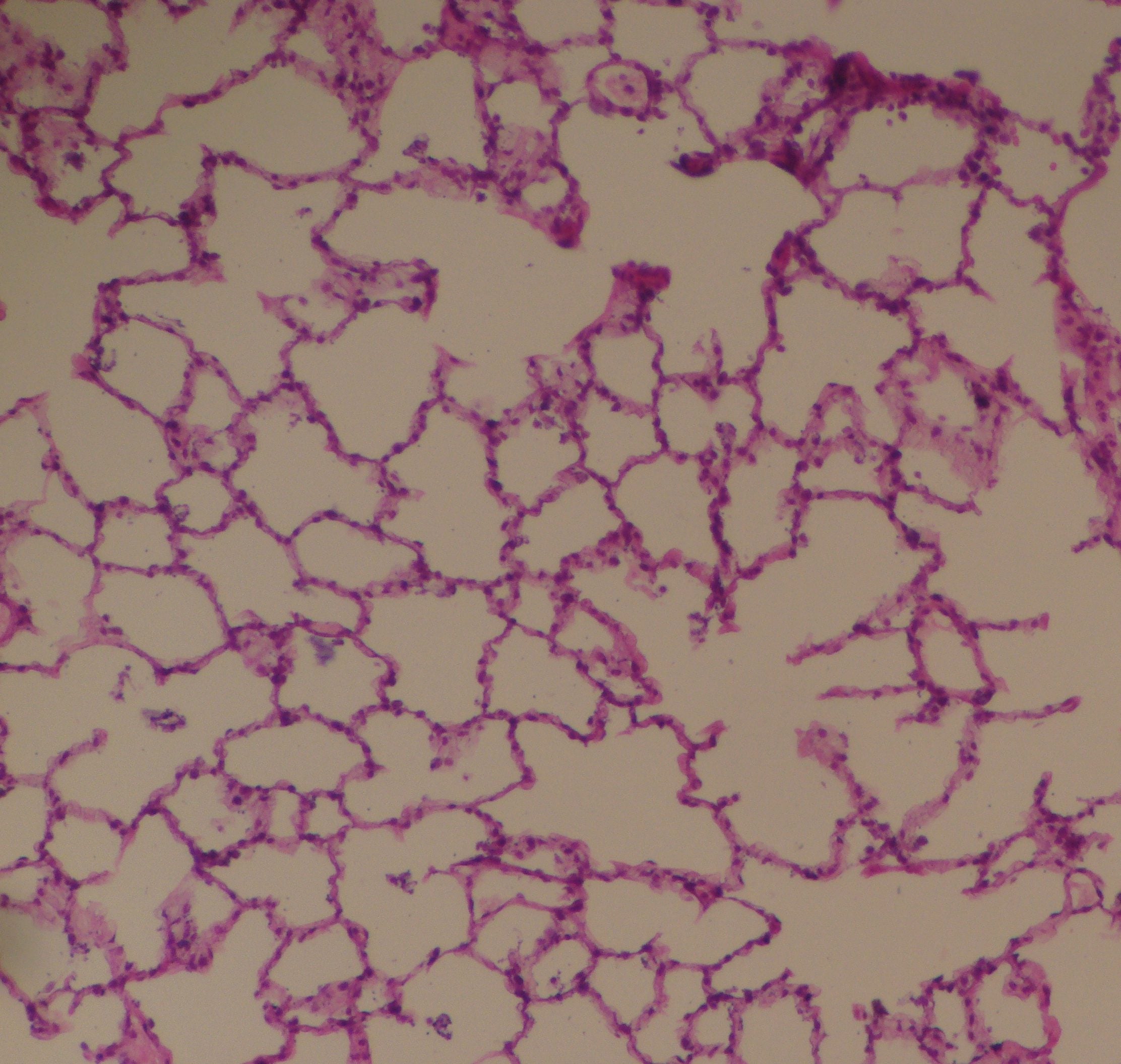
The lungs bring blood into near contact with the gas in the alveoli; only a very thin membrane separates them over a large surface area (Figure 4). Normally the contact lasts long enough that the gases carried in the blood leaving the alveoli are in equilibrium with the alveolar gas: the composition of the gas in the lungs determines the quantities of O2, CO2, and inert gas carried in arterial blood. Since whole-body arterial blood is the mixture of all the blood that returns to the heart from the lungs, PaCO2 is approximately equal to PACO2.
The balance between the rate of CO2 delivery from the blood and the rate of CO2 removal from the lungs by breathing sets PACO2. The rate of oxygen consumption (exercise intensity and heat generation) determines the rate of CO2 delivery to the lungs, while the rate at which the alveoli are “flushed” with fresh gas determines the rate of CO2 removal from the lungs. The rate of gas movement in and out of the alveoli is termed alveolar ventilation (V’A). PACO2 increases if V’A is too low for the rate of oxygen consumption.
Figure 4. A cross section of human alveoli.
https://commons.wikimedia.org/w/index.php?curid=46568489
The dark red dots are red blood cells. The open areas are the gas-filled alveoli, all of which connect to each other, but not necessarily in the plane of the thin slice. The blood vessels (pulmonary capillaries), each just wide enough for the red cells to squeeze through, are at right angles to the image.
CO2 diffuses constantly from the blood into the lung gas. Its concentration in lung gas decreases intermittently with the breathing cycle. The description of the instantaneous partial pressure in lung gas is thus very complex. However, if we consider a steady state situation and look at the average value across one minute, the rate at which breathing removes CO2 from the lungs (V’CO2) is
V’CO2 = V’A · (PACO2 – PiCO2) / k,
where PACO2 is average alveolar CO2 partial pressure over the minute, PiCO2 is CO2 partial pressure in the breathing gas, and the constant value k converts partial pressure to concentration in moles/volume using the ideal gas relationship.
V’A, defined above, is the rate at which gas enters and leaves the alveoli. Respiratory minute ventilation (V’E) is the rate at which gas enters and leaves the body through the nose and mouth. V’E is also the rate at which gas leaves or enters any breathing apparatus. (We will ignore the small differences in volume between inhaled and exhaled gas, differences primarily caused by temperature and humidity changes.) V’A is always less than V’E because V’E includes dead space ventilation. That is,
V’A = (VT – VD) ·f = V’E – (VD · f),
where VT is tidal volume, the volume of gas moved with each breath, which includes VD; VD is dead space volume, the total volume that contains expired gas at the start of inspiration, both that internal to the body (airways and other non-gas exchange regions of the lung) and that external to the body (contained in the breathing apparatus); and f is breathing frequency.
Thus, the average rate of CO2 removal from the lungs is
V’CO2 = (VT – VD) ·f · (PACO2 – PiCO2) / k,
A diver has some voluntary control of VT and f, but his or her respiratory control system (“Control of breathing”, below) usually determines the best combination. The manufacturer of the breathing gear has some control (within design constraints) of external VD and PiCO2. Both increase the volume of air that must be moved per minute to maintain a normal PACO2.
Another way to look at VD is as an increase in average PiCO2, since the amount of CO2 in any VT is
<(VD ·PACO2 + (VT-VD) · PiCO2>/ k,
and the average PiCO2 = <(VD ·PACO2 + (VT-VD) · PiCO2> / VT.
Thus, three factors increase the V’E necessary to maintain normal PACO2: exercise (rate of metabolic CO2 production), non-zero PiCO2, and larger than normal VD.
Control of breathing
The body senses chemical and physical conditions and modifies breathing patterns accordingly. (Of course, we also have short-term conscious control of our breathing patterns.)
Chemical control
All else being equal, the body’s sensors in the carotid arteries cause V’E to increase if PaCO2 is elevated or to decrease if PaCO2 is too low. Those on the surface of the brainstem respond similarly, but because they are bathed in cerebral spinal fluid rather than blood, they respond more slowly than those in the arteries to changes in PaCO2.
Physical control
Stretch When a person increases V’E from a resting, baseline level, both VT and f increase until stretch receptors limit further increases in VT. For greater V’E, VT stays constant and f increases.
Effort Although few textbooks mention it, respiratory muscles also appear to be protected against fatiguing work; if a breathing system provides external resistance, a person breathing on it decreases V’E even though PaCO2 increases. This happens even when the person can voluntarily increase V’E. The body appears to “accept” increasing PaCO2 to avoid excessive respiratory effort.12
Overall, the body adjusts V’E to balance at least two needs, that for a healthy PaCO2 and that to minimize respiratory effort.13 People breathing in closed spaces where they don’t need breathing gear (e.g., submariners) increase V’E to compensate for increased PiCO2 because their increase in respiratory effort is minimal. However, the greater the respiratory effort, the poorer the control of PaCO2. For example, subjects performing heavy exercise in a dry laboratory increased V’E and maintained nearly normal PETCO2 when PiCO2 was non-zero and external breathing resistance was minimal. However, they decreased V’E and let PETCO2 rise when moderate breathing resistance was added without inhaled CO2, and they responded minimally to non-zero PiCO2 when the moderate resistance was present, permitting PETCO2 to rise even higher. 3
Summary of factors affecting PACO2 and PaCO2
If only one factor changes (without adjustments by the control systems), PACO2 increases with the following:
Increasing | Decreasing |
exercise (metabolic rate) | V’E |
PiCO2 | |
VD |
Normal control without external respiratory loading increases V’E to hold PaCO2 normal during exercise and nearly normal with increased PiCO2 or VD. (Non-zero PiCO2 or large VD makes increases in V’E less effective.)
Normal control with resistive respiratory loading decreases V’E and lets PaCO2 rise even when the pressures necessary for higher V’E would be achieved under other conditions. This effect decreases the response to increased PiCO2 or VD, even to the point of eliminating it. With resistance in a breathing loop, increased PiCO2 or VD cause increased PaCO2. The amounts of resistance and PiCO2 and the volume of VD interact to determine whether or not the increase is a problem.
Current limits of acceptable external breathing resistance typically were generated with zero PiCO2 and low VD. Under those conditions, any increase in PaCO2 with a resistive breathing load is small. Unfortunately, the rise in PaCO2 associated with resistive loading is amplified by non-zero PiCO2, by large VD, or by exercise (including shivering). The risk is that some divers under those conditions have no symptoms of hypercapnia, while others have none until they feel panic or sudden severe distress.
Respiratory loading and VD can be made low by good equipment design, but they cannot be eliminated. Divers also face internal resistive loads caused by increased gas density at depth. Thus, non-zero PiCO2 poses a real risk, particularly when strenuous exercise is necessary. We cannot yet say what PiCO2 is acceptably safe for “normal” diving. We do know that if heavy exercise may be needed, 20 mbar (2 kPa, 2% SEV) is too much in the inspiratory loop, and that even 10 mbar (1 kPa, 1% SEV) is unwise.4
References
1. Respiratory Equipment – Self-contained Re-breathing Diving Apparatus, Brussels (Belgium): European Committee for Standardization; 2013, EN14143:2013 (E).
2. Respiratory equipment – Open-circuit Umbilical Supplied Compressed Gas Diving Apparatus – Part 2: Free Flow Apparatus, Brussels (Belgium): European Committee for Standardization; 2009, EN15333-2:2009 (E).
3. Shykoff BE and Warkander DE. “Exercise Carbon Dioxide (CO2) Retention with Inhaled CO2 and Breathing Resistance,” Undersea and Hyperbaric Medicine. Vol. 39, No. 4, pp. 815–828, July/Aug 2012.
4. Warkander DE and Shykoff BE. Combinations of Breathing Resistance and Inspired CO2: Effects on Exercise Endurance, NEDU TR 14-14, Navy Experimental Diving Unit, Feb 2015.
5. Xu F, Uh J, Brier MR, Hart J Jr, Yezhuvath US, Gu H, Yang Y, and Lu H. “The Influence of Carbon Dioxide on Brain Activity and Metabolism in Conscious Humans,” Journal of Cerebral Blood Flow & Metabolism. Vol. 31 No. 1, pp. 58–67, 2011.
6. Sayers JA, Smith REA, Holland RL, Keatinge WR. “Effects of Carbon Dioxide on Mental Performance,” J. Appl. Physiol., Vol. 63, No. 1, pp.25-30, 1967.
7. Otis AB, Rahn H, Epstein MA, and Fenn WO. “Performance as Related to Composition of Alveolar Air,” Am J Physiol. Vol. 146, No. 2, pp. 207–221, 1946.
8. Warkander DE, Norfleet WT, Nagasawa GK, and Lundgren CEG. ”CO2 Retention with Minimal Symptoms but Severe Dysfunction during Wet Simulated Dives to 6.8 atm abs.” Undersea Biomedical Research, Vol.17, No. 6, pp.515-523, 1990.
9. Selkirk A , Shykoff B, Briggs J. Cognitive Effects of Hypercapnia on Immersed Working Divers, NEDU TR 10-15, Navy Experimental Diving Unit, Oct 2010.
10. Arieli R, Shochat T, Adir Y. “CNS Toxicity in Closed-circuit Oxygen Diving: Symptoms Reported from 2527 Dives,” Aviation, Space, and Environmental Medicine, Vol. 77, No. 5, pp. 526–532, May 2006.
11. Foch AW. “Analysis of Recreational Closed-Circuit Rebreather Deaths 1998-2010,” Diving and Hyperbaric Medicine, Vol. 43, No. 2, pp.78-85, 2013.
12. Milic-Emili J and Tyler JM. “Relation between Work Output of Respiratory Muscles and End-Tidal CO2 Tension,” J. Appl. Physiol., Vol. 18, No. 3, pp. 663–671, 1963.
13. Poon CS. “Ventilatory Control in Hypercapnia and Exercise: Optimization Hypothesis,” J Appl. Physiol. Vol. 62, No. 6, pp.2447-2459, 1987.
---
Written by Barbara Shykoff
Barbara Shykoff earned her B.A.SC. (Engineering Science, Chemical option) from University of Toronto, her M.Sc.E (Bioengineering Institute) from the University of New Brunswick, and her Ph.D. (Biomedical Engineering Unit) from McGill University. For the last 16 years she has worked at the U.S. Navy Experimental Diving Unit. Her principal areas of investigation have been pulmonary oxygen toxicity, some other aspects of prolonged mildly-hyperoxic exposure, and physiological effects of breathing resistance with and without inspired CO2.
Written by Dan Warkander
Dan Warkander is an Engineer & Respiratory Physiologist who has worked with divers and their breathing equipment for over 30 years: air dives to 57 msw (190 fsw), Heliox dives to 450 msw (1500 fsw) and hydrogen-oxygen (hydrox) dives at 120 msw (400 fsw). He has led over 1,000 experimental dives; found and implemented breathing resistance limits for diving and dry-land use; developed a simple to use CO2 scrubber gauge.